Summer 2014
MATH 226: Limits and infinite series
Branko Ćurgus
- Wednesday, August 20, 2014
-
- Here is the
list of the topics for the final exam. Each of these topics might appear on the exam tomorrow.
- Tuesday, August 5, 2014
-
- Here is the
list of the topics that we covered after the first exam. Each of these topics might appear on the exam tomorrow.
- Tuesday, July 29, 2014
-
- This is an extended version of
Axioms for the Real Numbers. In this file I prove that our version of the Completeness Axiom (which is in fact taken from the book Mathematical analysis by Vladimir Zorich, translated by Roger Cooke,
published by Springer in 2004) is equivalent to the standard Completeness Axiom. As I mentioned in class, I prefer Zorich's version of the Completeness Axiom since it is stated using only the concepts introduced in preceding axioms. Moreover there is some symmetry in this axiom which is lacking in the standard version.
-
Today I proved that the sequence defined by
\[
S_n = \sum_{k=0}^n \frac{1}{k!}, \quad n \in {\mathbb N},
\]
converges. This sequence is often used to define the number $e$. However, as you can see on $e$-shirts, the sequence
\[
P_n = \biggl( 1 + \frac{1}{n}\biggr)^{\!n}, \quad n \in {\mathbb N},
\]
also converges to $e$. This is a classical result. I wrote a
short note with a proof of this result which will be published in The College Mathematics Journal at the beginning of 2015. In this
short note I prove the squeeze
\[
S_n - \frac{3}{2n} \leq P_n \leq S_n \qquad \text{for all} \qquad n \in {\mathbb N}.
\]
Since we already proved that the sequence $\{S_n\}$ converges, this squeeze and the Squeeze Theorem imply that the sequence $\{P_n\}$ also converges and the limit of $\{P_n\}$ equals to the limit of $\{S_n\}$.
- Monday, July 21, 2014
-
-
The next problem is not in the notes and it should be there. Use $\epsilon$-$\delta$ definition of continuity to prove that the function
\begin{equation*}
f(x) = \frac{x}{1-x^2}, \qquad -1 \lt x \lt 1,
\end{equation*}
is continuous on (-1,1).
-
Before proceeding with a proof notice that the following inequity holds
\[
1 - x^2 \geq 1-|x| \qquad \text{for all} \qquad x \in (-1,1).
\]
Call this inequality GI-1.
-
We need to prove that the function $f$ is continuous at each $a \in (-1,1)$. Let $a \in (-1,1)$ be arbitrary.
-
Let $x \in (-1,1)$ and simplify the expression
\begin{alignat*}{2}
\left| \frac{x}{1-x^2} - \frac{a}{1-a^2} \right|
& = \left| \frac{x(1-a^2) - a (1-x^2)}{(1-x^2)(1-a^2)} \right| & & \qquad \text{by algebra} \\
& = \left| \frac{(x-a)(1+xa)}{(1-x^2)(1-a^2)} \right| & & \qquad \text{by algebra} \\
& = \frac{|x-a| \ |1+xa|}{(1-x^2)(1-a^2)} & & \qquad \text{by abs rules, $1-x^2 \gt 0$, $1-a^2 \gt 0$} \\
& \leq \frac{|x-a| \, 1+|x|\ |a|}{(1-x^2)(1-a^2)} & & \qquad \text{by Triangle Inequality and abs rules} \\
& \leq \frac{2|x-a|}{(1-x^2)(1-a^2)} & & \qquad \text{since $|x| \lt 1$, $|a| \lt 1$} \\
& \leq \frac{2|x-a|}{(1-|x|)(1-|a|)} & & \qquad \text{by the inequality GI-1 above}
\end{alignat*}
Summarize:
\[
\left| \frac{x}{1-x^2} - \frac{a}{1-a^2} \right| \leq \frac{2|x-a|}{(1-|x|)(1-|a|)} \qquad \text{for all} \qquad x, a \in (-1,1).
\]
Call this inequality GI-2.
We need to simplify this further. For that we will consider two cases for $a$.
-
Case 1. $a \in [0,1)$.
(I) Take $\delta_0 = \frac{1-a}{2}$. Then $f(x)$ is defined for all $x \in \Bigl(a - \frac{1-a}{2}, a + \frac{1-a}{2} \Bigr) = \Bigl(\frac{3a-1}{2}, \frac{1+a}{2} \Bigr) \subset (-1,1)$.
(II) To continue the simplification from GI-2 we assume that $x \in \Bigl(\frac{3a-1}{2}, \frac{1+a}{2} \Bigr)$, that is $|x-a| \leq \frac{1-a}{2}$ and simplify GI-2 further
\begin{alignat*}{2}
\left| \frac{x}{1-x^2} - \frac{a}{1-a^2} \right|
& \leq \frac{2|x-a|}{(1-|x|)(1-|a|)} & & \qquad \text{by the inequality GI-2 above} \\
& \leq \frac{2|x-a|}{(1-\frac{1+a}{2})(1-|a|)} & & \qquad \text{since $x \in \Bigl(\frac{3a-1}{2}, \frac{1+a}{2} \Bigr)$ and $0 \leq a \lt 1$ we have $|x| \lt \frac{1+a}{2}$} \\
& = \frac{2|x-a|}{\frac{1-a}{2}(1-a)} & & \qquad \text{algebra and $a \geq 0$} \\
& = \frac{4|x-a|}{(1-a)^2} & & \qquad \text{algebra} \\
\end{alignat*}
-
Case 2. $a \in (-1,0)$.
(I) Take $\delta_0 = \frac{a+1}{2}$. Then $f(x)$ is defined for all $x \in \Bigl(a - \frac{a+1}{2}, a + \frac{a+1}{2} \Bigr) = \Bigl(\frac{a-1}{2}, \frac{1+3a}{2} \Bigr) \subset (-1,1)$.
(II)
To continue the simplification from GI-2 we assume that $x \in \Bigl(\frac{a-1}{2}, \frac{1+3a}{2} \Bigr)$, that is $|x-a| \leq \frac{a+1}{2}$ and simplify
\begin{alignat*}{2}
\left| \frac{x}{1-x^2} - \frac{a}{1-a^2} \right|
& \leq \frac{2|x-a|}{(1-|x|)(1-|a|)} & & \qquad \text{by the inequality GI-2 above} \\
& \leq \frac{2|x-a|}{(1-\frac{1-a}{2})(1-|a|)} & & \qquad \text{since $x \in \Bigl(\frac{a-1}{2}, \frac{1+3a}{2} \Bigr)$ and $-1 \lt a \lt 0$ we have $|x| \lt \frac{1-a}{2}$} \\
& = \frac{2|x-a|}{\frac{1+a}{2}(1-|a|)} & & \qquad \text{algebra} \\
& = \frac{2|x-a|}{\frac{1-|a|}{2}(1-|a|)} & & \qquad \text{since $a \lt 0$} \\
& = \frac{4|x-a|}{(1-|a|)^2} & & \qquad \text{algebra} \\
\end{alignat*}
-
Now we realize that the first and the second case can be unified:
(I) Take $\delta_0 = \frac{1-|a|}{2}$. Then $f(x)$ is defined for all $x \in \Bigl(a - \frac{1-|a|}{2}, a + \frac{1-|a|}{2} \Bigr) \subset (-1,1)$.
(II) Let $\epsilon \gt 0$ be arbitrary. To find $\delta(\epsilon)$ we need to solve
\[
\left| \frac{x}{1-x^2} - \frac{a}{1-a^2} \right| \lt \epsilon \qquad \text{for} \qquad |x-a|.
\]
To accomplish that summarize the inequalities from Case 1 and Case 2:
\[
|x-a| \leq \frac{1-|a|}{2} \qquad \Longrightarrow \qquad
\left| \frac{x}{1-x^2} - \frac{a}{1-a^2} \right| \leq \frac{4}{(1-|a|)^2}|x-a|.
\]
This is our BIN.
Now solve $\frac{4|x-a|}{(1-|a|)^2} \lt \epsilon$. The solution is $|x-a| \lt \frac{\epsilon (1-|a|)^2}{4}$.
Finally, set $\delta(\epsilon) = \min\left\{\frac{\epsilon (1-|a|)^2}{4}, \frac{1-|a|}{2} \right\}$.
It remains to prove
\[
|x-a| \lt \min\left\{\frac{\epsilon (1-|a|)^2}{4}, \frac{1-|a|}{2} \right\} \qquad \Longrightarrow \qquad \left| \frac{x}{1-x^2} - \frac{a}{1-a^2} \right| \lt \epsilon.
\]
Proving the last implication based on what has already been proven can be done as an exercise.
- Monday, July 14, 2014
-
- Here is the
list of the topics that we covered so far. Each of these topics might appear on the exam tomorrow.
- Wednesday, July 9, 2014
-
-
In this file I summarize steps involved in limit proofs for
.
-
Here is the Mathematica file that I created in class yesterday.
- Tuesday, July 1, 2014
-
-
Here I summarize steps involved in limit proofs for
.
- As a very simple example how to use Mathematica
here is the file that I created today.
- Another example of a Mathematica notebook is
here. In this Mathematica file I show how to explore functions in Mathematica. The file is called PlottingFunctions.nb. Right-click on the underlined word "Here"; in the pop-up menu that appears, your browser will offer you to save the file in your directory. Make sure that you save it with the exactly same name.
-
After saving the file you can open it with Mathematica. For this file use Mathematica 5.2. (We also have Mathematica 8 in BH 215. Ignore it for now.) You will find Mathematica 5.2 in most Windows computer labs on campus; click here for a list of labs with Mathematica installed (select Mathematica from the long list of programs and click search). To locate Mathematica on a particular computer you might try
Start -> All Programs -> Math Applications -> Mathematica.
Open Mathematica first; then open PlottingFunctions.nb from Mathematica. You can execute the entire file by the following manu sequence (in Mathematica):
Kernel -> Evaluation -> Evaluate Notebook.
There are some more instructions in the file.
-
To get started with Mathematica 5.2 see my
Mathematica page.
- As an introduction to the definition of a limit, we discussed the concept of a constant function.
- This is the definition that we gave in class: Let $A$ and $B$ be nonempty sets. A function $f: A \to B$ is a constant function if there exists $c \in B$ such that $f(x) = c$ for all $x \in A.$
- The negation of this definition is: Let $A$ and $B$ be nonempty sets. A function $f: A \to B$ is not a constant function if for every $c \in B$ there exists $x \in A$ such that $f(x) \neq c.$
- It is interesting to point out that the definition of a
constant function given on Wikipedia is slightly different: A function $f: A \to B$ is a constant function if for all $x, y \in A$ we have $f(x) = f(y).$
- The negation of the Wikipedia definition is: Let $A$ and $B$ be nonempty sets. A function $f: A \to B$ is not a constant function if there exist $x$ and $y$ in $A$ such that $f(x) \neq f(y).$
-
It is not difficult to prove that two definitions are equivalent. However, Wikipedia's definition is simpler.
- We also discussed the concept of an eventually constant function.
- Let ${\mathbb R}_+$ denote the set of all positive real numbers. A function $f: {\mathbb R}_+ \to {\mathbb R}$ is eventually constant if there exists $c \in {\mathbb R}$ and there exists $X \in {\mathbb R}_+$ such that $x \gt X$ implies $f(x) = c$.
- The negation of this definition is: A function $f: {\mathbb R}_+ \to {\mathbb R}$ is not eventually constant if for every $c \in {\mathbb R}$ and every $X \in {\mathbb R}_+$ there exists $x \gt X$ such that $f(x) \neq c$.
- The following item might help you in understanding mathematical statements involving quantifiers "for every" and "there exist" and negations of such statements.
- Consider grids which consist of black and white cells. For example consider the following four grids:
Grid 1 |
Grid 2 |
Grid 3 |
Grid 4 |
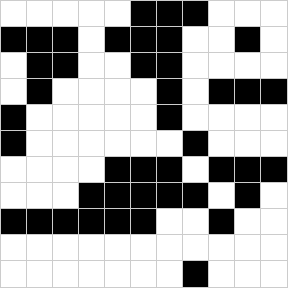 |
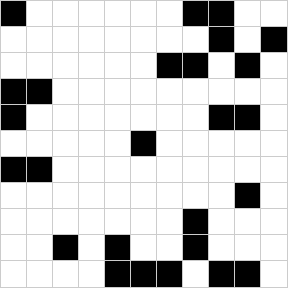 |
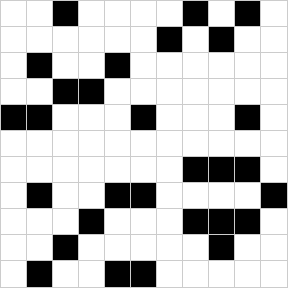 |
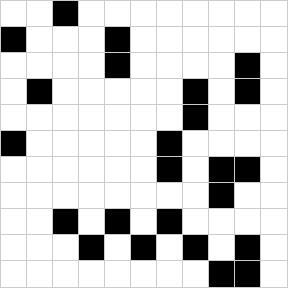 |
Further, consider the following four statements:
-
In every row there exists a black cell. (Statement 1)
-
There exists a column such that all its cells are white. (Statement 2)
-
There exists a row such that all its cells are white. (Statement 3)
-
In every column there exists a black cell. (Statement 4)
For each grid identify statements that are true for that grid. For each grid identify statements that are false for that grid. State the negations of each of the statements 1 through 4.
- Thursday, June 26, 2014
-
- Tuesday, June 24, 2014
-